AWS Quantum Technologies Blog
New research from Harvard and AWS will enable higher temperature operation of quantum communications networks
Following the announcement of a research alliance between the AWS Center for Quantum Networking and Harvard University, a joint team of Harvard and AWS scientists published a research paper today in Science Magazine discussing production of quantum memories that can operate at higher temperatures – enabling reduced cost and increased reliability for this fundamental component of quantum communication networks.
Quantum networks promise eavesdropper-proof communication by distributing entangled quantum bits (qubits) to network customers. Realizing this promise requires solving the distance limitations that plague state-of-the-art systems: entangled qubits communicated using photons over fiber-optic networks are lost after distances of around 100 miles. The distance problem can be solved through the use of satellite communications or with quantum repeaters, small quantum computers which can store and relay quantum bits.
Quantum repeaters extend the range of quantum communication through a process known as entanglement swapping. (See our companion blog post for greater detail). In this process, measurements performed on qubits at one repeater generate entanglement between qubits stored at other, more distant repeaters. This allows entanglement to be spread between repeaters that cannot communicate with each other directly, in turn enabling the transmission of quantum information over distances too long to be reliably traversed by a single photon.
In order to be useful in this capacity, a repeater must have efficient and high-fidelity interactions with individual photons, a memory time longer than the communication latency between the most distant parts of the network, the ability to perform operations between quantum bits stored within the same repeater, and the ability to be mass produced. The most difficult component of quantum repeaters to realize are devices that can catch and store photons, known as quantum memories. Quantum memories are still in their infancy, and a memory that could extend the range of networks was only demonstrated for the first time by a group at Harvard in 2020.
Many promising quantum memories suffer from degradation of their stored quantum bits (known as ‘decoherence’) if they aren’t operating at temperatures that are close to absolute zero. This requirement means that quantum repeaters need either helium dilution refrigerators, which are cumbersome and expensive, or complicated laser cooling systems to avoid decoherence driven by thermal vibrations, known as phonons.
A combined team of Harvard and AWS scientists worked with a leading quantum memory platform, silicon-vacancies in diamond crystals, and discovered how to reduce their temperature-related decoherence. For the silicon-vacancy in diamond, decoherence is driven by the interaction between the states which encode the quantum bit and phonons which start to appear in the diamond at a temperature of 1 kelvin (-272.15 C). Using strain in the diamond lattice, we were able to increase the energy of the transition between the qubit states so that it only interacted with higher energy phonons (which appear at 20 kelvin) – ensuring that no thermal phonons were able to drive this transition even at 4 kelvin. Operation at 4 kelvin is a commercially critical milestone because it enables a transition away from helium dilution refrigerators to more reliable and cost-effective technologies.
In the same paper, we also demonstrated quantum gates between the silicon-vacancy electron qubit (which interacts easily with its environment – including light) and qubit encoded in the spin of the silicon nucleus, which is less sensitive to environmental noise. (See Figure 1 for details.) Utilizing these interactions, we were able to demonstrate high-fidelity information exchange between light, the electron qubit, and the nuclear qubit. These results show that the silicon-vacancy has the ability to store and process multiple quantum bits at a time – one of the key requirements for a scalable quantum network.
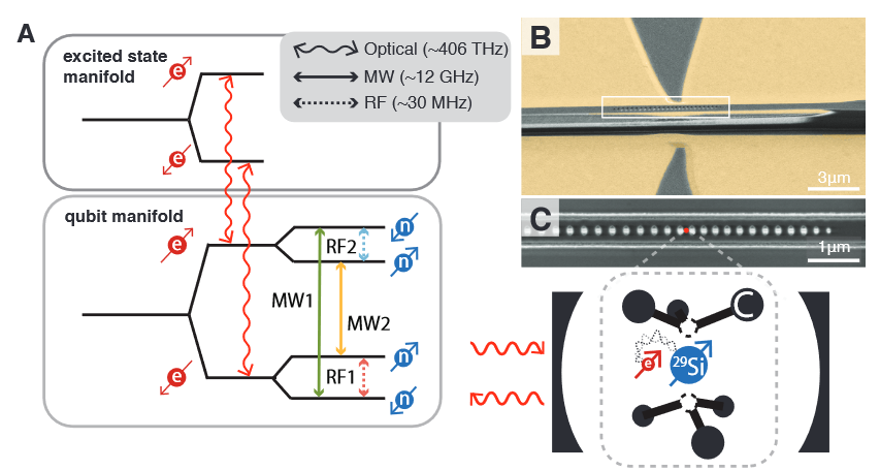
Figure 1: A figure from the Science paper, which outlines the interaction between photons, the silicon-vacancy electron qubit, and the nuclear qubit. A) The quantum levels of the silicon-vacancy center in diamond. The black lines indicate the energies of different states of the silicon vacancy. On the far left, without an external magnetic field, the silicon vacancy just has two energy levels, a ‘ground state’ and an ‘excited state’. If a laser hits the SiV, it will transition to the excited state and then emit a photon and jump back to the ground state. In the presence of a magnetic field (middle portion of the black lines), the ground and excited states take on different energies depending on the direction of the electron’s magnetic field. This magnetic field, known as the electron’s ‘spin’, encodes a quantum bit. On the far right, the effects of the nuclear qubit are added, which further shifts the energy up or down depending on the nuclear spin. Electrical control pulses, ‘MW’ and ‘RF’, can flip the nuclear and electron spins between up and down, allowing quantum operations between the quantum bit encoded on the electron and the quantum bit encoded in the nucleus. B) An image of the quantum memory device, taken using a scanning electron microscope. The white scale bar is 3 millionths of a meter (1/10,000 of an inch). The grey suspended material in the middle is a thin diamond wire, called a waveguide, that traps and guides light along its length. The yellow regions, surrounding it, are tiny wires made out of gold, which deliver the control pulses that change the state of the quantum bit. C) A zoomed in shot of the quantum memory device. The holes in the waveguide form mirrors that trap photons near the silicon-vacancy, allowing it to efficiently catch photons. The schematic below the image shows how the interaction with photons works: depending on the quantum state of the electron, the photons are reflected in different ways, enabling quantum information to be transferred from photons to the electron spin.
These advances pave the way for widely deployed, reliable quantum repeaters that will enable eavesdropper-proof communications and private access to quantum computers. Researchers at Harvard and AWS continue to work towards improving availability and quality of qubit host materials, improving the scalability of repeater hardware, and developing theoretical understanding of networks and different qubits that will be required to make this technology publicly available.